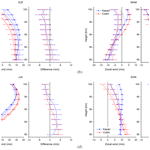
Influence of geomagnetic disturbances on mean winds and tides in the mesosphere/lower thermosphere at midlatitudes
Friederike Lilienthal
Dmitry Korotyshkin
Evgeny Merzlyakov
Gunter Stober
Observations of upper mesosphere/lower thermosphere (MLT) wind have been performed at Collm (51.3∘ N, 13.0∘ E) and Kazan (56∘ N, 49∘ E), using two SKiYMET all-sky meteor radars with similar configuration. Daily vertical profiles of mean winds and tidal amplitudes have been constructed from hourly horizontal winds. We analyse the response of mean winds and tidal amplitudes to geomagnetic disturbances. To this end, we compare winds and amplitudes for very quiet (Ap ≤ 5) and unsettled/disturbed (Ap ≥ 20) geomagnetic conditions. Zonal winds in both the mesosphere and lower thermosphere are weaker during disturbed conditions for both summer and winter. The summer equatorward meridional wind jet is weaker for disturbed geomagnetic conditions. Tendencies for geomagnetic effects on mean winds over Collm and Kazan qualitatively agree during most of the year. For the diurnal tide, amplitudes in summer are smaller in the mesosphere and greater in the lower thermosphere, but no clear tendency is seen for winter. Semidiurnal tidal amplitudes increase during geomagnetic active days in summer and winter. Terdiurnal amplitudes are slightly reduced in the mesosphere during disturbed days, but no clear effect is visible for the lower thermosphere. Overall, while there is a noticeable effect of geomagnetic variability on the mean wind, the effect on tidal amplitudes, except for the semidiurnal tide, is relatively small and partly different over Collm and Kazan.
- Article
(1117 KB) - Full-text XML
- BibTeX
- EndNote
It has long been known that not only the ionized upper atmosphere, but also the neutral middle atmosphere, is influenced by external drivers. The possibly most well known one is solar variability (e.g., Keuer et al., 2007; Beig, 2011; Stober et al., 2014; Qian et al., 2019), which influences the middle atmosphere on the 11-year cycle time scale, but also on shorter time scales like the 27 d solar rotation (e.g., Rong et al., 2020). Another factors are particle precipitation, which may influence the atmosphere from the lower thermosphere down to the surface (Mironova et al., 2015) in a more irregular manner and which is also related to geomagnetic variability. Model studies have shown that substorms lead to a loss of mesospheric ozone (Seppälä et al., 2015). As a consequence of ozone loss, mesospheric cooling has been observed (Pancheva et al., 2007).
The exact effect of geomagnetic activity on the neutral atmosphere dynamics to date is not fully quantified. During geomagnetic storms, in the upper thermosphere, enhanced high‐latitude energy and momentum inputs lead to strong Joule heating and ion drag, which change the neutral temperature and global circulation. At lower altitudes, observations have shown that winds also vary during storms. With the availability of radar observations in the mesosphere/lower thermosphere (MLT) region, the search for geomagnetic signatures has been performed based on these data, which are essentially horizontal winds. This has been done on the basis of case studies, epoch analyses (Singer et al., 1994), or in a more general statistical manner, i.e. in an attempt to quantify the influence of geomagnetic activity, often described by the Ap or Kp indices, on MLT winds (Fahrutdinova et al., 1999, 2001, 2013). The latter has also been done by including geomagnetic indices such as the Ap index in empirical relationships based on linear models of MLT wind variability (Pancheva et al., 2005). The response of the MLT to geomagnetic disturbances is not always clear. For example, Zhang et al. (2003) reported a different effect over Europe and Alaska, and related this to different effects during daytime and nighttime. This was also reported by Pancheva et al. (2007). Also Singer et al. (1994) revealed latitudinal differences of geomagnetic wind response. Recently, Yi et al. (2021) reported a clear effect of geomagnetic activity on zonal mean winds at high Antarctic latitudes, and especially an increase of tidal amplitude during disturbed days.
Several analyses have beeen performed to investigate geomagnetic activity effects on the MLT over Kazan (Fahrutdinova et al., 1999, 2001, 2013). Parameters that had been taken into account were mean winds, diurnal (DT) and semidiurnal (SDT) tides, and also irregular structures. While there was a relatively clear effect on the mean wind, particularly a decrease of the mesospheric jets with increasing geomagnetic activity, effects on tides and especially irregular structures (connected with gravity waves) seemed to be less clear.
In this paper, we will analyse more recent MLT winds from radar observations at Kazan, in an attempt to substantiate the earlier measurements in the literature. Furthermore, we will make use of the Collm radar at a similar latitude, in order to analyze common features and possible non-zonal structures in the reaction of the MLT to geomagnetic activity. We will, in addition to the DT and SDT, also include the terdiurnal tide (TDT) signature into the analysis. The TDT has been shown to be a non-negligible factor in MLT dynamics and has been analysed by several MLT radars already (Beldon et al., 2006; Jacobi, 2012; Jacobi and Fytterer, 2012; Liu et al., 2019).
At Collm (51.3∘ N, 13.0∘ E), a SKiYMET meteor radar is operated since summer 2004 (Jacobi et al., 2007). The radar operates on 36.2 MHz in a quasi all-sky configuration, and the main parameter observed are the MLT radial winds determined from the Doppler shift of individual meteor trails. Details of the radar system and the radial wind determination principle can be found in Jacobi (2012), Stober et al. (2012), and Lilienthal and Jacobi (2015). During 2015 the radar was upgraded by increasing the peak power to 15 kW, and replacing the until then used Yagi antennas by crossed dipoles, while maintaining the transmit frequency (Stober et al., 2018). The heights of the individual meteor trail reflections vary between about 75 and 110 km, and the maximum meteor count rate is found at an altitude slightly below 90 km (e.g., Stober et al., 2008).
The Kazan (56∘ N, 49∘ E) SKiYMET radar has been operating since 2015 (Korotyshkin et al., 2019b). This radar is equipped with a 15 kW transmitter, allowing high meteor rates and thus providing sufficient statistics for whole day observation in the height region 80–100 km. Transmitting frequency is 29.75 MHz. The transmitting antenna consists of two 3-element Yagis. The receiving system consists of five 2-elements Yagis. Collm and Kazan mean winds and SDT parameters have been analyzed by Korotyshkin et al. (2019a). They found a non-significant mean wind difference connected with stationary planetary waves and a slight difference in latitude. SDT differences are due to nonmigrating components.
The meteor radar hourly winds are computed applying a modified version of the so-called all-sky fit (Hocking et al., 2001; Stober et al., 2018). Daily mean winds have been calculated from these, and tidal amplitudes and phases have been calculated using the adaptive spectral filter described in Stober et al. (2017), Baumgarten and Stober (2019), and Stober et al. (2020). Note that from single radar measurements we cannot distinguish between tidal waves and other diurnal variations. We nevertheless use the notations DT, SDT, and TDT for the presentation of their amplitudes, because of their overall correspondence with the tidal amplitudes. We use total amplitudes calculated as the square-root of sum of the squared zonal and meridional amplitudes. We used data from August 2015, which is the beginning of the Kazan observations, to June 2020.
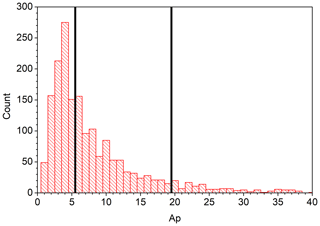
Figure 1Histogram of Ap indices during August 2015 and June 2020. The vertical lines show the chosen ranges for undisturbed and disturbed conditions.
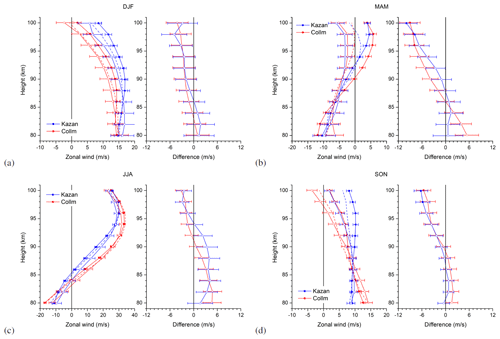
Figure 2Left plots: Long-term mean seasonal mean zonal mean winds during (a) DJF, (b) MAM, (c) JJA, (d) SON over Kazan (blue) and Collm (red) for undisturbed (Ap ≤ 5, solid symbols) and disturbed/unsettled (Ap ≥ 20, open symbols) conditions. Note the different scaling for the JJA wind profiles. Data are averages over 2015–2020. Error bars show standard errors. Wind profiles for 5 < Ap < 20 are added as dashed lines. The right plots in the respective panels show the differences for disturbed–undisturbed conditions. Solid symbols indicate differences >3 times the standard error.
To characterize the geomagnetic activity, daily Ap (GFZ, 2020) indices have been used. We characterize quiet conditions by Ap ≤ 5, which is actually a very strict requirement. Disturbed conditions are assumed to be connected with Ap ≥ 20, also including unsettled geomagnetic conditions. This resulted in a total of days between about 170 (in autumn) and 250 (winter/spring) for the undisturbed conditions and between about 30 (winter to summer) and 70 (autumn) for disturbed/unsettled conditions. The change with season is due to the annual/semiannual variation in geomagnetic activity (e.g., Le Mouël et al., 2004). A histogram of daily Ap indices during the time interval of the investigation is shown in Fig. 1. We calculated 2015–2020 mean seasonal 3-monthly means of the daily mean winds and tidal amplitudes for both conditions.
We present results for four seasons, namely winter (December–February, DJF), spring (March–May, MAM), summer (June–August, JJA) and autumn (September–November, SON). We present seasonal means of mean winds for disturbed and undisturbed conditions and their differences for both stations in Figs. 2 and 3.
Corresponding results for tidal amplitudes are shown in Figs. 4–6. However, particle precipitation and Joule heating at high latitudes depend on local time, and so it is possible that there is a local time dependent wind response, which could result in apparent changes in tidal amplitudes that should not be interpreted as geomagnetic activity effects on tidal waves. These effects cannot well be separated from a possible effect of mean wind changes on tidal wave propagation.
3.1 Mean winds
Figure 2 shows the 2015–2020 mean vertical profiles of the daily mean zonal wind for four seasons over Collm and Kazan for geomagnetically quiet and disturbed conditions on the respective left plots. The error bars show the standard error. On the right plots the differences between winds during disturbed and quiet conditions are shown together with their standard errors. Solid symbols indicate differences exceeding three times the standard error. Wind profiles for 5 < Ap < 20 are added as dashed lines on the left-hand plots. In most cases these values fall in the range between those for Ap ≤ 5 and those for Ap ≥ 20 and this is always the case for significant differences indicated by the solid symbols on the right-hand plots.
The zonal wind vertical profiles show the known seasonal cycle of zonal mean winds (e.g., Manson and Meek, 1984; Schminder et al., 1997; Jacobi, 2012; Korotyshkin et al., 2019a), with easterly mesospheric winds in spring/summer and a wind reversal in the lower thermosphere, and mesospheric westerlies in autumn/winter with an indication of the lower thermospheric wind reversal which is, however, generally seen above the height range considered. The differences between disturbed and undisturbed conditions are on the order of 5 m s−1, and they are positive (i.e. winds are more westerly during disturbed conditions) in the upper mesosphere and negative (more easterly winds during disturbed conditions) in the lower thermosphere throughout the year and qualitatively independent on season. Note that this leads to a weaker vertical wind gradient during disturbed conditions. This feature is mainly confirmed by Kazan radio meteor observations in the 1980s and 1990s (Fahrutdinova et al., 1999, 2001) and also corresponds to high-latitude observations reported by Yi et al. (2021). Note, however, that the differences are not significant in the mesosphere, and only present during MAM and SON in the lower thermosphere.
Arnold and Robinson (2001) modeled the response of the winter middle atmosphere on increased magnetic flux and found cooling in the polar stratosphere which leads to increased mesospheric zonal winds confirmed by our observations. Yi et al. (2018) observed decreasing density of the mid- to high-latitude mesosphere during geomagnetic storms, and proposed that this also influences mesospheric dynamics. Singer et al. (1994), however, have shown negative wind effects at about 90 km based on a number of different radar systems over Europe but, on the other hand, only weak effect over Siberia and a positive effect over Canada. This may be due to geomagnetic influences on the polar vortex, which may be shifted during geomagnetic storms (Hocke, 2017).
The meridonal winds in Fig. 3 are weaker, and generally not significant. They are southward in spring/summer and weak in winter as also known from earlier observations (Jacobi, 2012; Korotyshkin et al., 2019a). The differences are positive in spring and summer, i.e. the southward jet is weaker by about 4 m s−1 during disturbed conditions over both Collm and Kazan. This weaker meridional wind jet is connected with the weaker vertical zonal wind gradient (see Jacobi, 2012) and can be explained by weaker gravity wave filtering and resulting residual circulation in the case of a weaker mesospheric wind jet. During winter, the differences are again positive for Collm, but change sign with height over Kazan. During autumn the differences are weaker and differ over Collm and Kazan. The positive effect has already been shown by Fahrutdinova et al. (1999) for earlier Kazan observations, but has not been reported by Pancheva et al. (2005) from UK measurements at 90 km. On the other hand, Singer et al. (1994) reported weak geomagnetic effect on the mean wind.
3.2 Diurnal tide
Figure 4 shows the 2015–2020 mean vertical profiles of the daily mean total DT amplitude for four seasons over Collm and Kazan, again for geomagnetically quiet conditions in the respective left plots, and the differences between winds during disturbed and quiet conditions are shown in the respective right plots. Maximum amplitudes are found in summer at the upper altitudes. Smallest amplitudes are seen at about 85–90 km altitude, with increasing amplitudes below and above. This qualitatively confirms climatological results as in Manson et al. (2002), Jacobi (2012), and Xu et al. (2012), that also show the upper mesosphere maximum and the increasing amplitudes in the thermosphere. Note, however, that earlier climatologies often relied on vector averaging of tidal amplitudes or regression analyses including data from more than one day, which effectively reduces the analyzed amplitudes, so that the rather strong amplitudes at 80 km are often not that strong in these climatologies. Comparing the vertical structure with the one shown by Fahrutdinova et al. (1999, 2001) gives very good qualitative correspondence. Only their amplitudes are somewhat smaller in general, which may be owing to long-term tidal variability, or a change of the Kazan radar systems.
During disturbed conditions in summer and partly in spring, DT amplitudes in the upper mesosphere are somewhat decreased, but they are enhanced in the lower thermosphere. This feature is consistently seen over both Collm and Kazan. In autumn, DT amplitudes are reduced in the lower thermosphere, while there is no effect below approx. 90 km. In winter, the results for Collm and Kazan are contradicting. The results partly do not confirm the earlier ones by Fahrutdinova et al. (1999, 2001), who mainly found an increase of DT amplitudes for geomagnetically disturbed conditions.
3.3 Semidiurnal tide
Figure 5 shows the 2015–2020 mean vertical profiles of the daily mean SDT amplitude. Maximum amplitudes are seen in winter, and generally amplitudes increase with altitude above 85 km, as is known from the literature (see Schminder et al., 1997; Jacobi et al., 1999; Jacobi, 2012; Pokhotelov et al., 2018). As with the DT, the larger SDT amplitudes in the upper mesosphere are not well reproduced in early climatologies, owing to phase variability.
During winter, SDT amplitudes at altitudes above 85 km are significantly enhanced during geomagnetic disturbed days. This is also the case for the Collm amplitudes in spring, but not for Kazan during this season. A tendency for increasing amplitudes, although not seen at greater altitudes over Kazan, is also visible in summer. This was only weakly seen in earlier observations by Fahrutdinova et al. (1999). During spring and autumn, the SDT response to geomagnetic disturbances is unclear, and especially during spring it is different over the two stations. In the literature, also unclear or variable SDT response to geomagnetic variations has been reported (Singer et al., 1994).
3.4 Terdiurnal tide
Figure 6 shows the 2015–2020 mean vertical profiles of the daily mean TDT amplitude. Collm and Kazan profiles are very similar at 90 km altitude and above. Below, Kazan amplitudes are larger, indicating nonmigrating components of the tide. In winter, mean tidal amplitudes are somewhat larger than in summer, in agreement with literature (e.g. Beldon et al., 2006; Jacobi, 2012).
Differences between disturbed and undisturbed days are small and, except for spring at lower altitudes, on the order of 1 m s−1 only. In autumn, winter and spring, the amplitudes in the upper mesosphere are somewhat smaller for disturbed conditions. In the lower thermosphere, differences are small or, in autumn, the effects differ between Collm and Kazan. In spring and autumn the Collm upper mesosphere TDT amplitudes are generally smaller than the Kazan ones, and there is a tendency that they are stronger reduced for disturbed days than the Kazan ones. To summarize, TDT amplitudes are generally slightly reduced in the mesosphere during disturbed days, but no clear effect is visible for the lower thermosphere.
Joint observations of MLT winds have been performed at Collm (51.3∘ N, 13.0∘ E) and Kazan (56∘ N, 49∘ E) during 2015–2020 by two SKiYMET all-sky meteor radars with very similar configuration. Daily vertical profiles of mean winds and tidal amplitudes have been constructed from hourly horizontal winds. We analyze the response of mean winds and tidal amplitudes to geomagnetic disturbances and to this end compare winds and amplitudes for very quiet (Ap ≤ 5) and unsettled/disturbed (Ap ≥ 20) geomagnetic conditions.
Zonal winds in both the mesosphere and lower thermosphere are weaker during disturbed conditions for both summer and winter. Tendencies over Collm and Kazan for mean wind geomagnetic effects qualitatively agreed during most of the year. These results confirm earlier observations and are also supported by model studies. The summer equatorward meridional wind jet is weaker for disturbed geomagnetic conditions, as has already been shown from earlier observations at Kazan. We conclude that the geomagnetic effect on the mean wind is, for the region of our observations, a robust feature throughout the last decades, and may be explained by cooling/heating effects in the middle atmosphere and thermosphere. However, meridional wind observations reported by Pancheva et al. (2005) did not confirm the meridional wind effect.
The DT amplitudes in summer are smaller in the mesosphere but greater in the lower thermosphere. No clear tendency is seen for winter. SDT amplitudes increase during geomagnetic active days in both summer and winter. TDT amplitudes are sighly reduced in the mesosphere during disturbed days, but no clear effect is visible for the lower thermosphere. Overall, while there is a noticeable effect of geomagnetic variability on the zonal mean wind, our observed geomagnetic effect on tidal amplitudes is smaller and partly different over Collm and Kazan, which differs from results for high latitudes (Yi et al., 2021). It is also not clear of which nature a possible influence on tides could be, except for an indirect one. Modified background zonal winds could lead to changes in tidal propagation conditions. Given the weak or unsettled tidal response to geomagnetic disturbances this effect, however, seems to be small. Also, particle precipitation and Joule heating at high latitudes, depending on local time, may lead to a local time dependent wind response and therefore apparent changes in tidal amplitudes, which should not be interpreted as geomagnetic activity effects on tidal waves. These possible effects cannot be well separated from a possible effect of mean wind changes on tidal wave propagation.
Collm and Kazan radar wind data are available from the corresponding author on request. AP index data are available at GFZ Potsdam on ftp://ftp.gfz-potsdam.de/pub/home/obs/kp-ap/wdc/yearly/ (GFZ, 2020).
CJ initiated the study, provided the raw Collm data, and wrote the first draft of the manuscript. Raw Kazan data were provided by EM and DK. GS provided the mean wind and tidal analysis code. FL calculated daily wind and tidal amplitude profiles. All authors actively contributed to the writing of the paper.
The authors declare that they have no conflict of interest.
Publisher's note: Copernicus Publications remains neutral with regard to jurisdictional claims in published maps and institutional affiliations.
This article is part of the special issue “Kleinheubacher Berichte 2020”.
Ap indices have been kindly provided by GFZ Potsdam.
This research has been supported by the Deutsche Forschungsgemeinschaft (grant no. JA 836/38-1) and the Russian Foundation for Basic Research (grant no. 18-505-12048).
This paper was edited by Ralph Latteck and reviewed by three anonymous referees.
Arnold, N. F. and Robinson, T. R.: Solar magnetic flux influences on the dynamics of the winter middle atmosphere, Geophys. Res. Lett., 28, 2381–2384, https://doi.org/10.1029/2000GL012825, 2001. a
Baumgarten, K. and Stober, G.: On the evaluation of the phase relation between temperature and wind tides based on ground-based measurements and reanalysis data in the middle atmosphere, Ann. Geophys., 37, 581–602, https://doi.org/10.5194/angeo-37-581-2019, 2019. a
Beig, G.: Long-term trends in the temperature of the mesosphere/lower thermosphere region: 2. Solar response, J. Geophys. Res.-Space, 116, A00H12, https://doi.org/10.1029/2011JA016766, 2011. a
Beldon, C., Muller, H., and Mitchell, N.: The 8-hour tide in the mesosphere and lower thermosphere over the UK, 1988–2004, J. Atmos. Sol.-Terr. Phys., 68, 655–668, https://doi.org/10.1016/j.jastp.2005.10.004, 2006. a, b
Fahrutdinova, A., Sherstyukov, O., and Maksyutin, S.: The effect of geomagnetic activity on the dynamics of the upper mesosphere-lower thermosphere and on parameters of the Es-layer, Adv. Space Res., 24, 1499–1502, https://doi.org/10.1016/S0273-1177(99)00714-0, 1999. a, b, c, d, e, f, g
Fahrutdinova, A., Sherstyukov, O., and Maksyutin, S.: Geomagnetic activity influence on the dynamics of the upper mesosphere and lower thermosphere, Int. J. Geomag. Aeron., 2, 201–208, 2001. a, b, c, d, e
Fahrutdinova, A., Maksyutin, S., and Elakhov, M.: Effects of sector structure of the interplanetary magnetic field on the upper mesosphere–lower thermosphere dynamics, Adv. Space Res., 52, 1959–1965, https://doi.org/10.1016/j.asr.2013.08.031, 2013. a, b
GFZ: Indices of Global Geomagnetic Activity, available at: ftp://ftp.gfz-potsdam.de/pub/home/obs/kp-ap/wdc/yearly/, last access: 27 August 2020. a, b
Hocke, K.: Response of the middle atmosphere to the geomagnetic storm of November 2004, J. Atmos. Sol.-Terr. Phys., 154, 86–91, https://doi.org/10.1016/j.jastp.2016.12.013, 2017. a
Hocking, W., Fuller, B., and Vandepeer, B.: Real-time determination of meteor-related parameters utilizing modern digital technology, J. Atmos. Sol.-Terr. Phys., 63, 155–169, https://doi.org/10.1016/S1364-6826(00)00138-3, 2001. a
Jacobi, C.: 6 year mean prevailing winds and tides measured by VHF meteor radar over Collm (51.3∘ N, 13.0∘ E), J. Atmos. Sol.-Terr. Phys., 78–79, 8–18, https://doi.org/10.1016/j.jastp.2011.04.010, 2012. a, b, c, d, e, f, g, h
Jacobi, C. and Fytterer, T.: The 8-h tide in the mesosphere and lower thermosphere over Collm (51.3∘ N; 13.0∘ E), 2004–2011, Adv. Radio Sci., 10, 265–270, https://doi.org/10.5194/ars-10-265-2012, 2012. a
Jacobi, C., Portnyagin, Y., Solovjova, T., Hoffmann, P., Singer, W., Kashcheyev, B., Oleynikov, A., Fahrutdinova, A., Solntsev, R., Beard, A., Mitchell, N., Muller, H., Schminder, R., and Kürschner, D.: Mesopause region semidiurnal tide over Europe as seen from ground-based wind measurements, Adv. Space Res., 24, 1545–1548, https://doi.org/10.1016/S0273-1177(99)00878-9, 1999. a
Jacobi, C., Fröhlich, K., Viehweg, C., Stober, G., and Kürschner, D.: Midlatitude mesosphere/lower thermosphere meridional winds and temperatures measured with meteor radar, Adv. Space Res., 39, 1278–1283, https://doi.org/10.1016/j.asr.2007.01.003, 2007. a
Keuer, D., Hoffmann, P., Singer, W., and Bremer, J.: Long-term variations of the mesospheric wind field at mid-latitudes, Ann. Geophys., 25, 1779–1790, https://doi.org/10.5194/angeo-25-1779-2007, 2007. a
Korotyshkin, D., Merzlyakov, E., Jacobi, C., Lilienthal, F., and Wu, Q.: Longitudinal MLT wind structure at higher mid-latitudes as seen by meteor radars at central and Eastern Europe (13∘ E/49∘ E), Adv. Space Res., 63, 3154–3166, https://doi.org/10.1016/j.asr.2019.01.036, 2019a. a, b, c
Korotyshkin, D., Merzlyakov, E., Sherstyukov, O., and Valiullin, F.: Mesosphere/lower thermosphere wind regime parameters using a newly installed SKiYMET meteor radar at Kazan (56∘ N, 49∘ E), Adv. Space Res., 63, 2132–2143, https://doi.org/10.1016/j.asr.2018.12.032, 2019b. a
Le Mouël, J.-L., Blanter, E., Chulliat, A., and Shnirman, M.: On the semiannual and annual variations of geomagnetic activity and components, Ann. Geophys., 22, 3583–3588, https://doi.org/10.5194/angeo-22-3583-2004, 2004. a
Lilienthal, F. and Jacobi, Ch.: Meteor radar quasi 2-day wave observations over 10 years at Collm (51.3∘ N, 13.0∘ E), Atmos. Chem. Phys., 15, 9917–9927, https://doi.org/10.5194/acp-15-9917-2015, 2015. a
Liu, H., Tsutsumi, M., and Liu, H.: Vertical structure of terdiurnal tides in the Antarctic MLT region: 15-year observation over Syowa (69∘ S, 39∘ E), Geophys. Res. Lett., 46, 2364–2371, https://doi.org/10.1029/2019GL082155, 2019. a
Manson, A. and Meek, C.: Winds and tidal oscillations in the upper middle atmosphere at Saskatoon (52∘ N, 107∘ W, L=4.3) during the year June 1982–May 1983, Planet. Space Sci., 32, 1087–1099, https://doi.org/10.1016/0032-0633(84)90134-X, 1984. a
Manson, A. H., Meek, C., Hagan, M., Koshyk, J., Franke, S., Fritts, D., Hall, C., Hocking, W., Igarashi, K., MacDougall, J., Riggin, D., and Vincent, R.: Seasonal variations of the semi-diurnal and diurnal tides in the MLT: multi-year MF radar observations from 2–70∘ N, modelled tides (GSWM, CMAM), Ann. Geophys., 20, 661–677, https://doi.org/10.5194/angeo-20-661-2002, 2002. a
Mironova, I. A., Aplin, K. L., Arnold, F., Bazilevskaya, G. A., Harrison, R. G., Krivolutsky, A. A., Nicoll, K. A., Rozanov, E. V., Turunen, E., and Usoskin, I. G.: Energetic particle influence on the Earth's atmosphere, Space Sci. Rev., 194, 1–96, https://doi.org/10.1007/s11214-015-0185-4, 2015. a
Pancheva, D., Mukhtarov, P., Mitchell, N., and Muller, H.: Empirical model of the dynamics in the mesosphere and lower thermosphere region over the UK, including solar and geomagnetic activity, J. Atmos. Sol.-Terr. Phys., 67, 197–209, https://doi.org/10.1016/j.jastp.2004.07.029, 2005. a, b, c
Pancheva, D., Singer, W., and Mukhtarov, P.: Regional response of the mesosphere–lower thermosphere dynamics over Scandinavia to solar proton events and geomagnetic storms in late October 2003, J. Atmos. Sol.-Terr. Phys., 69, 1075–1094, https://doi.org/10.1016/j.jastp.2007.04.005, 2007. a, b
Pokhotelov, D., Becker, E., Stober, G., and Chau, J. L.: Seasonal variability of atmospheric tides in the mesosphere and lower thermosphere: meteor radar data and simulations, Ann. Geophys., 36, 825–830, https://doi.org/10.5194/angeo-36-825-2018, 2018. a
Qian, L., Jacobi, C., and McInerney, J.: Trends and solar irradiance effects in the mesosphere, J. Geophys. Res.-Space, 124, 1343–1360, https://doi.org/10.1029/2018JA026367, 2019. a
Rong, P., von Savigny, C., Zhang, C., Hoffmann, C. G., and Schwartz, M. J.: Response of middle atmospheric temperature to the 27 d solar cycle: an analysis of 13 years of microwave limb sounder data, Atmos. Chem. Phys., 20, 1737–1755, https://doi.org/10.5194/acp-20-1737-2020, 2020. a
Schminder, R., Kürschner, D., Singer, W., Hoffmann, P., Keuer, D., and Bremer, J.: Representative height-time cross-sections of the upper atmosphere wind field over Central Europe 1990–1996, J. Atmos. Sol.-Terr. Phys., 59, 2177–2184, https://doi.org/10.1016/S1364-6826(97)00062-X, 1997. a, b
Seppälä, A., Clilverd, M. A., Beharrell, M. J., Rodger, C. J., Verronen, P. T., Andersson, M. E., and Newnham, D. A.: Substorm-induced energetic electron precipitation: Impact on atmospheric chemistry, Geophys. Res. lett., 42, 8172–8176, https://doi.org/10.1002/2015GL065523, 2015. a
Singer, W., Bremer, J., Hoffmann, P., Manson, A., Meek, C., Schminder, R., Kürschner, D., Portnyagin, Y., Makarov, N., Muller, H., Kazimirovsky, E., and Clark, R.: Geomagnetic influences upon tides—winds from MLT radars, J. Atmos. Terr. Phys., 56, 1301–1311, https://doi.org/10.1016/0021-9169(94)90068-X, 1994. a, b, c, d, e
Stober, G., Jacobi, C., Fröhlich, K., and Oberheide, J.: Meteor radar temperatures over Collm (51.3∘ N, 13∘ E), Adv. Space Res., 42, 1253–1258, https://doi.org/10.1016/j.asr.2007.10.018, 2008. a
Stober, G., Jacobi, C., Matthias, V., Hoffmann, P., and Gerding, M.: Neutral air density variations during strong planetary wave activity in the mesopause region derived from meteor radar observations, J. Atmos. Sol.-Terr. Phys., 74, 55–63, https://doi.org/10.1016/j.jastp.2011.10.007, 2012. a
Stober, G., Matthias, V., Brown, P., and Chau, J. L.: Neutral density variation from specular meteor echo observations spanning one solar cycle, Geophys. Res. Lett., 41, 6919–6925, https://doi.org/10.1002/2014GL061273, 2014. a
Stober, G., Matthias, V., Jacobi, C., Wilhelm, S., Höffner, J., and Chau, J. L.: Exceptionally strong summer-like zonal wind reversal in the upper mesosphere during winter 2015/16, Ann. Geophys., 35, 711–720, https://doi.org/10.5194/angeo-35-711-2017, 2017. a
Stober, G., Chau, J. L., Vierinen, J., Jacobi, C., and Wilhelm, S.: Retrieving horizontally resolved wind fields using multi-static meteor radar observations, Atmos. Meas. Tech., 11, 4891–4907, https://doi.org/10.5194/amt-11-4891-2018, 2018. a, b
Stober, G., Baumgarten, K., McCormack, J. P., Brown, P., and Czarnecki, J.: Comparative study between ground-based observations and NAVGEM-HA analysis data in the mesosphere and lower thermosphere region, Atmos. Chem. Phys., 20, 11979–12010, https://doi.org/10.5194/acp-20-11979-2020, 2020. a
Xu, X., Manson, A., Meek, C., Riggin, D., Jacobi, C., and Drummond, J.: Mesospheric wind diurnal tides within the Canadian Middle Atmosphere Model Data Assimilation System, J. Atmos. Sol.-Terr. Phys., 74, 24–43, https://doi.org/10.1016/j.jastp.2011.09.003, 2012. a
Yi, W., Reid, I. M., Xue, X., Murphy, D. J., Hall, C. M., Tsutsumi, M., Ning, B., Li, G., Younger, J. P., Chen, T., and Dou, X.: High- and middle-latitude neutral mesospheric density response to geomagnetic storms, Geophys. Res. Lett., 45, 436–444, https://doi.org/10.1002/2017GL076282, 2018. a
Yi, W., Reid, I. M., Xue, X., Murphy, D. J., Vincent, R. A., Zou, Z., Chen, T., Wang, G., and Dou, X.: First observations of Antarctic mesospheric tidal wind responses to recurrent geomagnetic activity, Geophys. Res. Lett., 48, e2020GL089957, https://doi.org/10.1029/2020GL089957, 2021. a, b, c
Zhang, S. P., Salah, J. E., Mitchell, N., Singer, W., Murayama, Y., Clark, R. R., van Eyken, A., and Thayer, J.: Responses of the mesospheric wind at high latitudes to the April 2002 space storm, Geophys. Res. Lett., 30, 2225, https://doi.org/10.1029/2003GL018521, 2003. a